

Hydrogel interfaces for merging humans and machines
source link: https://www.nature.com/articles/s41578-022-00483-4
Go to the source link to view the article. You can view the picture content, updated content and better typesetting reading experience. If the link is broken, please click the button below to view the snapshot at that time.
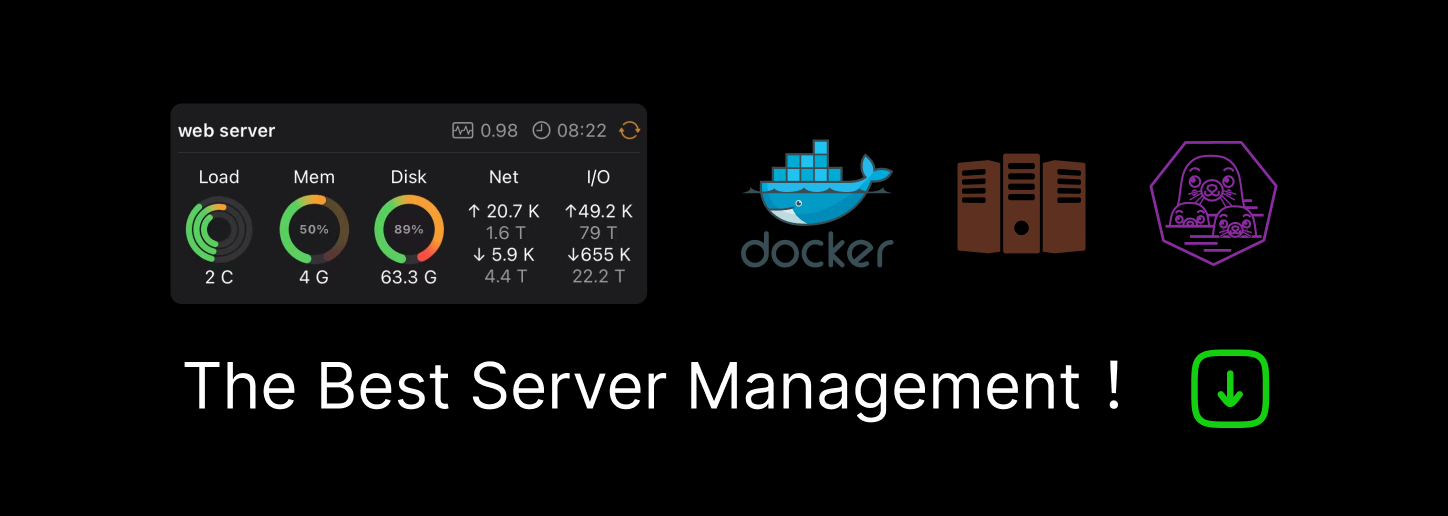
Introduction
In the last century, advances in the understanding and engineering of the human body were made possible through interdisciplinary efforts in modern medicine, biology and biomedical engineering. In parallel, complex and ever more capable machines, such as computers, mobile devices, sensors, actuators and robots, have transitioned from science fiction into daily realities. Despite these advances, artificial interfaces — facilitating communication and interactions between humans and machines — are still largely primitive, leading to short-term and inefficient interfacing between humans and machines. These shortcomings are especially apparent in the emerging fields of brain–machine interfaces, neuroprosthetics, clinical equipment, medical implants, wearable and ingestible devices, and virtual or augmented reality. Long-term, efficient, biocompatible and seamless communication and interactions between humans and machines could lead to breakthroughs in these emerging sectors and other technological fields but have not been achieved due to lingering challenges. For example, conventional probes and arrays used for brain–machine interfaces and neuroscientific studies, such as the Michigan probes and Utah arrays, commonly induce substantial foreign-body responses, such as gliosis and scar formation, severely hampering the long-term reliability and functionality of brain–machine interfaces1,2,3,4,5,6. As another example, implantable glucose sensors and insulin pumps for diabetic monitoring and management often face substantially limited efficacy in the long term owing to fibrotic encapsulation induced by a foreign-body response and subsequent loss of the functional interfaces for sensing and delivery to the surrounding tissues7,8,9,10,11,12.
The main challenges of human–machine interfaces stem from materials. Existing machines largely employ conventional materials, such as metals, silicon, glass, ceramics and plastics, to communicate and interact with human bodies. However, the hard, dry and abiotic nature of these conventional materials is intrinsically contradictory with the soft, wet and living nature of biological tissues. In recent decades, intensive efforts have been devoted to transforming these conventional materials into flexible and stretchable structures to conformally interface with soft and curvilinear biological tissues1,13,14,15,16,17. However, these structural designs do not alter the materials’ intrinsic properties, which may still hamper their communication and interactions with biological tissues. For example, the integration of conventional materials with tissues usually relies on physical attachment or surgical suturing, methods that face challenges such as non-conformal contact, unstable adhesion, tissue damage and/or scar formation15,18,19. As another example, the properties of metallic electrodes, such as their high rigidity, low interfacial capacitance and low charge injection capacity, make them far from ideal for the electrical recording and stimulation of soft neural tissues1,2,5,17,20,21,22. In addition, biological tissues often recognize these materials, even when in flexible and stretchable structures, as foreign bodies due to their inherently disparate properties, resulting in an adverse foreign-body response, biofouling, and fibrotic encapsulation or fibrosis2,23,24. Such foreign-body response and subsequent fibrotic isolation from the surrounding tissues can severely compromise the long-term reliability and efficacy of the communication and interactions between humans and machines10,23,25,26,27,28,29.
Because of their unique similarities to biological tissues30,31,32,33 (Box 1) and the versatility and flexibility in tailoring their properties5,33,34,35,36,37, hydrogels have naturally emerged as a promising material candidate to act as an alternative or adjunct to conventional materials for bridging humans and machines. Hydrogel interfaces — both explored in academic research and as commercial products — are rapidly emerging in a broad range of applications (Fig. 1). For instance, ultrasound-coupling hydrogels have been commercialized and have become the standard interfaces between ultrasound equipment and human skin for various medical imaging and therapeutic applications37,38,39,40,41,42. Similarly, commercially available skin-adhesive hydrogels have become the main candidates for epidermal electrodes used in clinical bioelectronic recordings, such as in electrocardiography, electromyography (EMG) and electroencephalography, and in clinical bioelectronic stimulation such as transcutaneous electrical nerve stimulation5,41,42,43,44,45. Besides their application as ultrasound-coupling agents and bioelectrodes, hydrogels have also been intensively explored as sensors, actuators and drug reservoirs in wearable devices such as sweat sensors46,47,48, contact lenses49,50,51,52, and wound dressings and bandages53,54,55,56. In addition, taking advantage of their edible, food-like properties and tunable swelling and degradation properties, hydrogels have recently emerged as a promising carrier for ingestible sensors and devices capable of long-term retention and functions in the gastrointestinal tract57,58,59. Furthermore, hydrogels with designed mechanical properties and chemical compositions have been shown to substantially enhance the biocompatibility of implantable devices by providing highly lubricious surfaces60,61,62,63,64,65, reducing biofouling64,66,67,68 and alleviating foreign-body responses69,70,71,72,73,74,75,76,77,78. This enhanced biocompatibility paves the way for various implantable devices to form long-term reliable and functional interfaces with the human body.
Various examples of hydrogel interfaces for epidermal and wearable (part a), implantable (part b), and ingestible and minimally invasive (part c) applications around the human body. FBR, foreign-body response.
Despite the great promise and recent advances in hydrogel interfaces, to the best of our knowledge, there is no systematic discussion on hydrogel interfaces for the merging of humans and machines. The literature on hydrogels as scaffolds for tissue engineering31,32,79,80,81,82,83, carriers for drug delivery84,85,86,87 and emerging materials for soft machines34,88 has been extensively reviewed, but existing reviews usually do not account for the applications of hydrogels as bridging interfaces between humans and machines nor do they provide the requirements or principles for the design of hydrogel interfaces. Such a systematic discussion is central for the future development of this nascent yet impactful field.
In this Review, we first provide a comprehensive summary of recent advances in hydrogel interfaces using examples from both academic literature and commercial products. Based on the nature of the communication and interactions between humans and machines, the functions of hydrogel interfaces can be broadly categorized into mechanical, acoustic, electrical, optical, chemical and biological modes. Thereafter, we systematically discuss the property requirements for hydrogel interfaces to enable various functional modes and provide the design principles to achieve such properties. We conclude with future perspectives on next-generation hydrogel interfaces and the remaining challenges and opportunities.
Box 1 Similarities between hydrogels and biological tissues
High water content
The human body contains few organs with a relatively low water content (such as bone, ~25% of weight) and many organs with a high water content (such as muscle, ~70% of weight, and brain, ~85% of weight), resulting in an average water content of ~70% of body weight270 (see the figure, part a). By contrast, most engineering materials, such as silicon, metals, ceramics, plastics and elastomers, are dry and contain little or no water5. Because water in biological tissues serves critical roles in diverse biological, chemical and physical processes, including nutrient and waste transportation, intercellular signalling by waterborne chemicals, and ionic electrophysiological communication, hydrogels with a tissue-like high water content can closely mimic physiological interactions and minimize potential issues caused by dry foreign materials.
Mechanical softness
The human body is largely composed of soft tissues with few exceptions such as teeth and bone. Soft tissues in the human body have Young’s moduli typically in the range of 1 Pa to 1 MPa, whereas most conventional solids, such as elastomers, plastics, inorganics and metals, exhibit Young’s moduli several orders of magnitude higher2,13 (see the figure, part b). This stark difference in mechanical properties is one of the major sources of tissue damage, foreign-body response and scar tissue formation around the rigid devices interfacing with the human body1,2,3,5. By contrast, hydrogels can have Young’s moduli similar to those of biological tissues owing to their structural and compositional similarities as highly hydrated polymer networks.
Biocompatibility
Biocompatibility is one of the most crucial requirements for interfacing with the human body while avoiding adverse biological interactions and outcomes. Owing to their non-toxic and biocompatible nature, hydrogels have been widely studied and adopted in tissue engineering31, regenerative medicine29,32 and biomedical devices41 in both academic research and commercial products. Furthermore, hydrogels can offer antifouling64,66,67,68 functions and reduce foreign-body responses69,70,71,72,73,74,75,76,77,78 when in contact with physiological environments.
Biofunctionality
Both biological tissues and hydrogels are water-based polymer systems, and a broad range of biopolymers and synthetic polymers with biologically important functional groups can be used as constituents for hydrogels31,33,80,81. For example, various extracellular matrix components (such as collagen, gelatin and hyaluronic acid) as well as chemically modified biopolymers and synthetic polymers (such as arginyl-glycyl-aspartic acid (RGD) peptide-modified alginate and polyethylene glycol) have been adopted in hydrogels to facilitate biological functionalities (such as cell adhesion253 and tissue engineering29,31).

GI, gastrointestinal; PMMA, poly(methyl methacrylate); PETE, poly(ethylene terephthalate).
Applications of hydrogel interfaces
Along with various devices and machines in contact and interacting with human bodies, hydrogel interfaces have emerged and have been adopted in diverse applications in both academic and commercial settings (Table 1). The current applications of hydrogel interfaces can be largely categorized based on the invasiveness of their interactions with various parts of the human body (Fig. 1). Hydrogel interfaces for epidermal and wearable applications (Fig. 1a) provide non-invasive communication, whereas hydrogel interfaces for implantable applications (Fig. 1b) are invasive and often provide long-term contact with internal organs and tissues. Hydrogel interfaces are also increasingly adopted in ingestible and minimally invasive applications within body cavities (such as thoracic and abdominal cavities) or tubular organs (such as intestines and blood vessels) (Fig. 1c). In this section, we review current applications of hydrogel interfaces from each category using examples from the literature and commercially available products (Tables 1 and 2).
Epidermal and wearable applications
Skin is the largest organ in the human body and forms the body’s outermost interface to external environments15. The facile and non-invasive nature of epidermal interfacing has encouraged the development of a wide range of epidermal and wearable devices, many of which have been commercialized and adopted as the standard of care in clinical practice. Despite its environment-exposed nature, skin possesses starkly different properties than conventional materials used in devices and machines. To bridge these dissimilarities and allow stable functionality, diverse hydrogel interfaces have been adopted in epidermal and wearable applications (Fig. 1a).
Epidermal electrodes are an essential component for various electrical sensing and stimulation devices in health monitoring15, diagnostic5,15, therapeutic45,89 and human–machine interfacing90 applications. The efficacy of epidermal electrical communications is influenced by several factors, including conformal mechanical contact with skin and the degree of skin hydration5,43,91. Conventional metallic electrodes are dry and mechanically stiff; therefore, they face various limitations, such as poor interfacial contact with dehydrated skin tissues, resulting in high skin–electrode interfacial impedance and ineffective electrical communication5. To address these limitations, hydrogel interfaces were introduced as coatings on metal electrodes and have become the standard electrode coatings in various clinical diagnostic (such as electrocardiography, electromyography and electroencephalography) and therapeutic (such as transcutaneous electrical nerve stimulation) applications. The soft and adhesive properties of hydrogel epidermal electrodes provide conformal contact with skin, and their high water content and high electrical conductivity offer low tissue–electrode impedance, synergistically leading to effective electrical sensing and stimulation. Notably, the majority of commercially available and clinically approved epidermal electrodes are composed of ionic hydrogel interfaces consisting of crosslinked hydrophilic polymers, such as polyacrylate copolymers, which have a high water content, good skin adhesiveness and dissolved ions (for example, potassium chloride) for electrical conductivity5,92 (Table 2).
Furthermore, epidermal diagnostic imaging techniques, such as ultrasonography, rely on hydrogel couplants at the tissue–ultrasound probe interface, owing to the tissue-like mechanical and acoustic characteristics of hydrogels38,93,94,95,96. Hydrogel interfaces have also been widely adopted for the treatment of injured skin in the form of wound dressings or epidermal bandages owing to their unique capabilities for promoting wound healing through dehydration prevention, antimicrobial protection and transdermal drug delivery53,55,56,97,98,99 (Table 2). In addition, hydrogel interfaces have been used in many epidermal health monitoring and diagnostic applications100. For example, the high water absorption of various chemical-sensing hydrogels enables their use as diagnostic devices based on epidermally collected body fluids such as sweat51,101,102,103,104,105,106,107.
Beyond skin, hydrogel interfaces have been widely utilized in ophthalmic wearable devices to interface with the eye. The soft, wet and transparent nature of hydrogels and their optical properties, such as their tunable refractive index, are highly desirable for ophthalmic interfacing. The majority of contact lenses, including clinically approved products based on poly(2-hydroxyethyl methacrylate) (Acuvue, J&J) and polyvinyl alcohol hydrogels (Dailies, CIBA Vision) (Table 2), have been based on hydrogels since the first commercial launch of hydrogel-based soft contact lenses in the 1970s108,109. More recently, various health monitoring and diagnostic functions have been introduced to ophthalmic hydrogel interfaces to create smart contact lenses49,50,51,52,108,110.
Implantable applications
In order for devices or machines to interface over the long term with internal tissues and organs, they often need to be implanted into the human body. Unlike non-invasive epidermal interfacing, implantable applications involve invasive surgical procedures and introduction of foreign objects within the human body. As a result, implantable applications face various challenges, including tissue damage, foreign-body responses and subsequent functional failure of the implants. These challenges often originate from and are substantially exacerbated by disparate properties between biological tissues and the implants. Hydrogel interfaces have been adopted for diverse implantable applications to resolve or alleviate these challenges (Fig. 1b).
The surgical repair of injured tissues and implantation of devices on targeted organs commonly requires establishing robust mechanical interfaces between the injured tissues and between the devices and organs, respectively. Conventional techniques to form mechanical interfaces, such as sealing and fixation, rely on sutures and surgical staples111. However, sutures and surgical staples commonly damage tissues, and their substantial mismatch with tissue properties can cause various adverse outcomes and complications, including scar formation, impaired healing and leakages112. In recent decades, tissue adhesives and sealants have been developed to address the limitations of sutures and surgical staples113,114,115. Because hydrogels provide robust, biocompatible and tissue-matching adhesive interfaces, a broad range of hydrogels based on synthetic polymers116,117 (such as polyethylene glycol) and biopolymers118,119 (such as fibrin or gelatin) have been adopted in implantable tissue adhesives for tissue repair114 and integrated in devices both in academic studies and in clinically approved products19 (Table 2).
Implantable electrodes for neurological treatment (such as deep brain stimulation)120,121,122, for rehabilitation (such as spinal cord stimulation)123,124,125,126,127 and for functional augmentation involving, for example, pacemakers128,129,130,131, cochlear implants132 or neuroprosthetics2,133,134,135, require reliable bioelectronic communication and modulation over extended periods of time. However, conventional metallic implantable electrodes can elicit adverse responses such as fibrosis and scar formation from the target and surrounding tissues, resulting in functional loss owing to a decrease in signal-to-noise ratios during recording and a decrease in charge injection capacity during stimulation2,4,6,21. To address these issues, hydrogel interfaces have been introduced as adjunct or alternatives to metallic electrodes to provide improved biocompatibility69,76,136,137 due to their tissue-matching Young’s modulus and lower bending stiffness as well as improved electrical properties5,77,78,138,139 resulting from their lower impedance and higher charge injection capacity.
Optical interfacing has also been utilized in implantable applications for photomedicine in photothermal therapy, photodynamic therapy and photobiomodulation110 as well as for the modulation of neural activities in optogenetics21,140, where optical communication with the target tissue commonly relies on implanted waveguides110. However, conventional optical waveguides are made of silica or plastics and their mechanical mismatch with biological tissues can cause various adverse outcomes and deterioration of functionalities110. To avoid these limitations, hydrogel-based implantable optical waveguides with tissue-matching mechanical properties have been developed as a promising alternative to conventional stiff optical waveguides110,141,142,143,144.
Sustained delivery of pharmacological substances from implanted reservoirs is one of the major strategies for the treatment of various diseases145. Hydrogels have played an important role in the development and translation of implantable drug delivery systems owing to their favourable characteristics, including high water content, ease of chemical modification and incorporation of drugs, biocompatibility, and biodegradability84,87,146. As a result, various hydrogels, such as poly(2-hydroxyethyl methacrylate), hyaluronic acid and alginate, have been adopted in clinically approved products as implantable drug reservoirs or tissue scaffolds31,42,87 (Table 2). In implantable applications, such as for sustained drug delivery, the foreign-body response and subsequent blockage of drug release by biofouling and fibrosis around the implanted devices is a critical issue hampering long-term efficacy9. To alleviate this challenge, diverse anti-foreign-body response hydrogel interfaces have been adopted in implantable machines and devices in academic studies to minimize the formation of fibrotic encapsulation (by reducing protein absorption and inflammatory reactions) and to preserve long-term functional efficacy64,66,67,68,147.
Minimally invasive applications
Interfacing within body cavities, such as abdominal and thoracic cavities, and tubular organs, such as the intestines, blood vessels and the urinary tract, provides minimally invasive yet close interactions with the human body. Orally administered ingestible devices have been developed for diverse diagnostic and therapeutic gastrointestinal applications59. Recent advances in endoscopic and robot-assisted surgical platforms have enabled a rapid growth in minimally invasive and robotic surgeries148. Despite the minimally invasive nature of these applications, close interactions and communication with the internal tissues and organs require biocompatible and benign interfacing. Various hydrogel interfaces have been incorporated in a wide range of ingestible and minimally invasive devices to offer more favourable tissue–device interactions and communication (Fig. 1c).
Taking advantage of the soft, biocompatible and highly swellable characteristics of hydrogels, several hydrogel-based gastrointestinal-retentive ingestible devices have been developed for health monitoring58,149, drug delivery57 and weight control150, including several clinically approved products such as weight control devices based on cellulose hydrogels (Table 2). Swollen hydrogels have similar mechanical properties to food, providing favourable mechanical interactions with the gastrointestinal organs during their administration, retention and digestive passage from the body.
Endoluminal insertion and navigation of catheters, guidewires and stents are a routine part of minimally invasive surgeries and daily patient care. However, mechanical interactions of the inserted devices with narrow tubular organs can cause complications such as frictional tissue damage and infection. To alleviate these issues, various hydrogels have been introduced in clinically approved catheters (in particular, polyvinylpyrrolidone hydrogels), guidewires (in particular, poly(methyl vinyl ether/maleic anhydride) hydrogels) and stents (in particular, poly(methyl vinyl ether/maleic anhydride) hydrogels) in the form of thin coatings to provide soft, low-friction and antifouling interfacing with the endoluminal surface of tubular organs64,151,152,153,154,155,156 (Table 2).
Functional modes of hydrogel interfaces
Machines and the human body can interact and communicate via diverse functional modes depending on the desired applications and the characteristics of the target tissues and organs. Hence, hydrogel interfaces bridging machines and the human body also need to incorporate a wide range of functional modes. We classify the functional modes of hydrogel interfaces into six categories: mechanical, acoustic, electrical, optical, chemical and biological (Table 3 and Fig. 2).
Various functional modes of communication and interactions between humans and machines via hydrogel interfaces, including mechanical (part a), acoustic (part b), electrical (part c), optical (part d), chemical (part e) and biological (part f) modes and the desired properties of hydrogel interfaces for each mode. σtissue, conductivity of the tissue media; AP, action potential; Ce, capacitance of the hydrogel interface; FBR, foreign-body response; IAP, target cell’s transmembrane current amplitude of action potential; Iinjection, current injected to the target tissue by electrophysiological stimulation; r, distance between the electrode and the target cell; Re, resistance of the hydrogel interface; Ric, interconnect resistance; Vrecording, electric potential of the electrophysiological recording; Vstimulation, applied electric potential for electrophysiological stimulation.
In addition, because a machine can interface with the human body via multiple functional modes simultaneously, hydrogel interfaces often need to be multifunctional (Table 1). For example, epidermal electrodes require hydrogel interfaces to function not only in the electrical mode through electrical conduction but also in the mechanical mode exploiting skin-matching mechanical properties and adhesiveness5,157. Therefore, the rationally guided design and development of materials for hydrogel interfaces necessitate the systematic consideration of various functional modes and the corresponding desired properties to enable multifunctional applications. In this section, we discuss the six major functional modes of hydrogel interfaces and the desired properties for each functional mode.
Mechanical mode
The mechanical mode of hydrogel interfaces is essential because it guarantees their integrity and robustness as well as their stable adhesion and matching rigidity with the target tissues and organs. Hydrogel interfaces in the mechanical mode are required to possess a set of bulk and interfacial properties (Fig. 2a and Table 3).
Many machines and devices require prolonged contact with the human body. For example, wearable devices for health monitoring form close contact with the epidermis for days to weeks. Long-term implantable devices can stay within the body contacting internal tissues and organs over months to years. The various tissues and organs in the human body possess substantially different mechanical stiffness. Mechanical interactions between the target tissues or organs and hydrogel interfaces with dissimilar mechanical stiffness can cause impaired functional efficacy, for example, through non-conformal contact, as well as long-term adverse tissue responses such as foreign-body response, tissue damage or scar formation2,33,88,114. Therefore, a desired bulk property of a hydrogel interface is a mechanical stiffness, or Young’s modulus, matching that of the target tissue or organ.
The human body is a dynamic system mechanically interacting with external environments and within itself. Hence, hydrogel interfaces also face highly dynamic mechanical interactions with the human body. Preventing bulk failures caused by mechanical loads and deformation is a key design criterion to ensure the reliable functionality of hydrogel interfaces. Thus, a high fracture toughness is another desirable bulk property for hydrogel interfaces158,159,160,161.
Various applications of hydrogel interfaces, such as epidermal electrodes, tissue adhesives and sealants, require interfacial integration with the target tissues to provide the desired functionality (Table 1). Interfacial integration of hydrogel interfaces with the human body requires establishing and maintaining robust adhesion to biological tissues. Adhered hydrogel interfaces can undergo interfacial failures, which can result in functional loss, for example, through the detachment of devices, or detrimental clinical complications such as leakage from failed tissue sealants. To achieve robust interfacial integration, hydrogel interfaces require high interfacial toughness116,117,162,163,164,165.
Another important function of hydrogel interfaces in the mechanical mode is the reduction of friction and wear with the target tissues and organs. Interfacial mechanical interactions between hydrogel interfaces and the target tissues and organs can cause frictional wear. Sustained frictional wear can cause the interfacial erosion of poorly lubricated hydrogel interfaces and/or tissue damage. Hence, a low friction coefficient is desirable to minimize frictional wear in applications such as minimally invasive medical devices64,154 and artificial cartilage65,166.
Acoustic mode
Owing to its non-destructive and non-invasive nature, the sound wave-based diagnosis of diseases and health conditions has become standard clinical practice94. Furthermore, the capability of ultrasound to deliver energy into deep tissues in a non-destructive manner has been utilized in various therapeutic applications, including thermal treatments and non-thermal therapies exploiting mechanical effects, such as ultrasonic lithotripsy96, as well as in drug delivery167,168. Hence, the acoustic mode of hydrogel interfaces can be exploited for two types of applications: acoustic imaging by facilitating the delivery of sound waves to and reflection from the target, such as in ultrasonography, and acoustic stimulation by facilitating the delivery of sound waves to the target such as in ultrasonic lithotripsy (Fig. 2b).
Ultrasonic transducers and probes used for these applications are made of conventional materials with an acoustic impedance substantially different from that of skin33. Furthermore, the high mechanical rigidity of the ultrasonic transducers and probes prevents their conformal interfacial contact with skin, resulting in air-filled gaps that also have disparate acoustic impedance compared to skin. The resultant mismatch in acoustic impedance at the skin–probe interface can severely hinder the transmission of acoustic waves owing to interfacial reflections and scattering169, deteriorating functional efficacy (for example, decreasing the quality of sonographic images). Hence, tissue-matching acoustic impedance and conformal interfacial contact with skin are advantageous for hydrogel interfaces that work in the acoustic mode93,169,170 (Fig. 2b and Table 3).
Electrical mode
Machines can communicate and interact with electrically active tissues and organs in the human body, such as the brain, nerves, muscles and heart, through hydrogel interfaces functioning in the electrical mode5. The electrical mode of hydrogel interfaces can be used in two types of applications based on the direction of electrical communication: from the human body to hydrogel interfaces for electrophysiological recording, and from hydrogel interfaces to the human body for electrophysiological stimulation (Fig. 2c and Table 3).
Based on a simplified equivalent circuit model5 (Fig. 2c), the electrical potential in electrophysiological recording and the injected current in electrophysiological stimulation can be quantitatively expressed as:
where σtissue is the conductivity of the tissue, r is the distance between the electrode and the target cell, Re is the resistance of the hydrogel interface, s is the complex frequency of the action potential (for recording) or the stimulation waveforms (for stimulation), Ce is the capacitance of the hydrogel interface, Ric is the interconnect resistance, IAP is the target cell’s transmembrane current amplitude of action potential, Iinjection is the current injected to the target tissue by the electrophysiological stimulation, and Vstimulation is the applied electric potential for electrophysiological stimulation5.
In electrophysiological recording, a high Vrecording is desirable. Hence, for given physiological (σtissue, r, s, IAP) and recording (Ric) conditions, high electrical conductivity (low Re) and high capacitance (high Ce) are advantageous. Notably, physiological environments exhibit native ionic conductivity (0.3–0.7 Sm-1)19,171 because of the high water content of biological tissues (Box 1) and their rich ionic compositions comprised of salts and charged proteins. Hence, the electrical conductivity of hydrogel interfaces should be substantially higher than that of the surrounding tissue to ensure the quality of recorded signals5,172,173.
In electrophysiological stimulation, a high Iinjection, often measured as charge injection capacity, is desirable. Hence, for a given stimulation device (Ric) and input (Vstimulation), high electrical conductivity (low Re) and high capacitance (high Ce) are advantageous. Therefore, high electrical conductivity and high capacitance are desirable properties of hydrogel interfaces in the electrical mode for both recording and stimulation applications.
Optical mode
The optical mode of hydrogel interfaces relies on the transmission and delivery of light to the human body (Fig. 2d and Table 3). Unblocked transmission of incident light to the eye is a critical requirement for visual functionality and eyesight. Hence, hydrogel interfaces for ophthalmic applications require high transmittance to minimize the attenuation of transmitted light to the eye108,110. Beyond ophthalmic interfacing, the delivery of a broad spectrum of light to diverse tissues and organs is enabling the use of hydrogel interfaces for photonic diagnosis and treatment110. Light delivery requires an optical pathway typically in the form of a waveguide that provides directional guidance for the transmitted light, where the transmittance and refractive index respectively modulate the transmission and internal reflection of light143,144. Therefore, hydrogel interfaces for light delivery require high transmittance and a tunable refractive index141,142,174.
Chemical mode
Physiological activities involve diverse chemical processes and reactions and, therefore, chemical interactions with the human body are one of the key functional modes of hydrogel interfaces. The high water content of biological tissues and hydrogel interfaces inherently allows the exchange of waterborne chemicals. Based on the direction of the chemical communication, the chemical mode of hydrogel interfaces can be further divided into two types: from hydrogel interfaces to the human body for chemical delivery, and from the human body to hydrogel interfaces for chemical sensing (Fig. 2e and Table 3).
Chemical delivery is commonly utilized for the administration of pharmacological substances, such as drugs and other biologics, for therapeutic interventions and treatments84,86,87,145. The therapeutic efficacy and toxicity of drugs are highly sensitive to their dosage and release profile; therefore, the capability to engineer controlled release from hydrogel interfaces is a critical requirement145. Chemicals within hydrogel interfaces can be released to the surrounding tissue by diffusion or by degrading the hydrogel. The rate and profile of chemical delivery by diffusion are determined by the specific diffusivity of the chemicals in the hydrogel84, whereas the rate and profile of chemical delivery by hydrogel degradation rely on the biodegradability of the hydrogel84. Because different drugs have different sizes and interactions with hydrogel matrices, tunable mesh sizes, interactions with chemicals (such as binding and release) and/or biodegradability are highly desirable for chemical delivery87.
The sensing of a certain chemical species requires selectivity in interactions between the target chemical and hydrogel interfaces146,175,176,177,178,179. This selectivity can be controlled by tuning the binding affinity to the target chemical as a higher binding affinity provides a higher selectivity177. Hence, a high binding affinity to target chemicals is desirable for selective chemical sensing.
Biological mode
Interfaces between machines and biological tissues inherently involve a broad range of biological interactions that substantially influence the machines’ efficacy, reliability and biosafety. As a result, the biological mode of hydrogel interfaces is of essential importance, especially in applications that require higher invasiveness and long-term interactions with the human body such as implantable devices. The biological mode of hydrogel interfaces can be divided into various types depending on the application requirements: promotion of biological activities (such as cell adhesion, proliferation, infiltration and differentiation) and suppression of biological activities (such as anti-foreign-body responses)180 (Fig. 2f and Table 3).
Cell adhesion and infiltration to hydrogel interfaces and subsequent partial or full remodelling of the hydrogel interfaces by native tissues are favourable for various implantable applications. For example, implanted devices for drug delivery and tissue repair (such as tissue adhesives and sealants) require the gradual infiltration of cells from the surrounding tissue with ultimate resorption and tissue remodelling to avoid the need for surgical removal of the devices and potential long-term adverse effects such as chronic inflammation87,114,181. Cellular interactions and tissue remodelling processes rely on the initial attachment of cells to the hydrogel, which is followed by the progressive degradation of the hydrogel and infiltration of cells31,35,81,182,183. Hence, high cell adhesiveness and biodegradability are important in applications where tissue remodelling is desired.
Regulating unfavourable biological interactions between the implanted device and the surrounding tissue is also an important function of hydrogel interfaces. Biofouling and foreign-body response in physiological environments and the resultant fibrosis can be detrimental to the long-term efficacy of hydrogel interfaces4,23. Because fibrotic encapsulation and scar tissues possess much lower electrical conductivity and chemical diffusivity than native tissues, they substantially reduce the efficacy of electrical sensing and stimulation2,3,4,5,173,184 as well as of chemical delivery and sensing9,23,185,186,187,188. Therefore, a low foreign-body response is highly desirable for efficient long-term communication in the electrical, chemical and optical modes28,185,188.
Hydrogel–machine interfaces
In addition to hydrogel–tissue interfaces, interfaces between hydrogels and machines are also important. To avoid interfacial failure between hydrogels and machines, hydrogel–machine interfaces require robust integration with high interfacial toughness162,163. For the acoustic, electrical and optical modes of hydrogel interfaces, the robust integration between hydrogels and machines should also provide high acoustic transmittance, high optical transparency162 and high electrical conductivity139, respectively. In addition, chemical delivery and sensing can be performed by a machine, such as a drug reservoir or a sensor coated in the hydrogel, instead of by the hydrogel itself. In this case, a high permeability or diffusivity of the chemicals at the hydrogel–machine interface is required to allow unhindered transportation of chemicals between the target tissue and the machine87. This Review focuses primarily on hydrogel–tissue interfaces but several reviews are available that discuss hydrogel–machine interfaces33,34,88.
Design principles for hydrogel interfaces
Enabling the functional modes of hydrogel interfaces requires the implementation of various desired hydrogel properties (Table 3). Although hydrogels can offer diverse properties, achieving optimal properties for a desired functional mode is complex33,37. As a result, Edisonian approaches based on trial and error have limited success5,33, particularly for the development of multifunctional hydrogel interfaces.
In this section, we summarize rational design principles to achieve desired properties for hydrogel interfaces, providing some examples from the literature (Table 3 and Fig. 3).
Rational design principles to achieve desired properties for hydrogel interfaces, including mechanical properties such as high fracture toughness36,158,159 (part a), high interfacial toughness162,163,209 (part b) and low friction coefficient212 (part c); electrical properties such as high electrical conductivity5,217,218,219,223 (part d) and high capacitance5,77,221 (part e); chemical properties such as tunable diffusivity230,231,232 (part f) and tunable biodegradability181,269 (part g); and biological properties such as a low foreign-body response (FBR)28,70,73,75,188,258 (part h). The grey dotted boxes provide an enlarged view of selected areas. ξ, mesh size of the hydrogel polymer network; CNT, carbon nanotube; H-bond, hydrogen bond; PAni, polyaniline; PEDOT, poly(3,4-ethylenedioxythiophene); PPy, polypyrrole; rs, radius of the chemical.
Mechanical properties
Mechanical interactions between hydrogel interfaces and the human body are affected by the hydrogel bulk and interfacial mechanical properties, including Young’s modulus, fracture toughness, interfacial toughness and friction coefficient (Fig. 2a). Because the mechanical properties of tissues are determined by their compositional and structural features, the design principles for the mechanical properties of hydrogel interfaces are largely based on mimicking the target tissue compositional and/or structural characteristics.
Soft tissues (which include skin, muscle, nerve and internal organs) have relatively low Young’s moduli (1 kPa to 10 MPa) owing to their crosslinked extracellular matrices (Box 1). Conventional hydrogels consisting of crosslinked polymer networks show compositional and structural similarities with soft tissues, including low Young’s moduli33. The Young’s moduli of conventional hydrogels can be tuned by controlling the density of polymer chains per unit volume, n, as follows:
where k is the Boltzmann constant and T is the absolute temperature33; n can be engineered by varying the polymer concentration and the crosslink density of the hydrogel33. Hence, diverse conventional hydrogels with varying compositions (for example, GelMA hydrogels189,190, alginate hydrogels191,192,193 or chitosan hydrogels194,195,196) have been adopted in hydrogel interfaces to provide tissue-matching Young’s moduli.
Stiff connective tissues, such as tendons and ligaments, exhibit significantly higher (10 MPa to 1 GPa) and anisotropic (stiffer in the longitudinal direction) Young’s moduli than soft tissues. These properties largely originate from their highly aligned fibrous microstructures197. Several strategies using synthetic polymers such as polyvinyl alcohol and biopolymers such as alginate, silk and cellulose197,198,199,200,201 have achieved aligned fibrous hydrogels that are tendon-like. Bone tissues show very high Young’s moduli (1–30 GPa) owing to the rich mineral contents of their main component, hydroxyapatite202. Hence, several bone-matching hydrogel interfaces have been developed by incorporating a high concentration of hydroxyapatite within the hydrogel matrix203,204.
Notably, additional considerations can arise for specific applications. For instance, hydrogel interfaces with a Young’s modulus higher or lower than that of the target tissue can influence cellular responses via mechanotransduction, a mechanism that modulates various biological processes205 such as cell migration (for example, fibroblast migration towards a substrate with higher Young’s modulus), proliferation or differentiation (for example, promoted osteoblast differentiation on a substrate with higher Young’s modulus). Moreover, beyond Young’s modulus, rate-dependent mechanical properties, such as viscoelasticity and poroelasticity, can be beneficial for certain applications, including tissue engineering83,206 and bioelectronics78,207, to match the mechanical properties of the target tissue.
To achieve high fracture toughness, mechanical dissipation and stretchability must be synergistically combined33,36,208 (Fig. 3a). The resultant fracture toughness can be expressed as follows:
where Γ0 is the intrinsic fracture toughness and ΓD is the contribution of the mechanical dissipation in the process zone (the region ahead of the crack tip that dissipates mechanical energy) to the total fracture toughness36,159. Because the intrinsic fracture toughness of conventional hydrogels is commonly limited to 10–100 Jm-2, high mechanical dissipation is needed for high fracture toughness36. The mechanical dissipation of a hydrogel within the process zone is proportional to the area of the hysteresis loop in its stress–stretch curve during a loading–unloading cycle (Fig. 3a). Various strategies to provide high mechanical dissipation have been developed, such as using double-network hydrogels158,159 (hydrogels consisting of two interpenetrating networks) and nanocomposite hydrogels160,161 (hydrogels containing nanoscale crosslinkers), to introduce sacrificial networks and bonds into hydrogels.
To achieve high interfacial toughness for hydrogels adhered to tissues, high fracture toughness and strong interfacial linkages between the hydrogel and tissue need to be synergistically integrated33,162 (Fig. 3b). The resultant interfacial toughness can be expressed as follows:
where Γ0inter is the intrinsic interfacial toughness from the interfacial linkages and ΓDinter is the contribution of the mechanical dissipation in the process zone162,209. High intrinsic interfacial toughness can be achieved by strong interfacial linkages between the hydrogel and the tissue. Mechanisms that provide strong interfacial linkages between tough dissipative hydrogels and biological tissues or machines include covalent bonds19,117,210 (such as amide bonds), high-density hydrogen bonds211 (such as in dry-annealed polyvinyl alcohol hydrogels) and topological interpenetration of polymers116,164 (such as in topologically interpenetrating chitosan solutions).
By making the hydrogel surface non-adhesive, low friction coefficients between hydrogels and tissue surfaces can be achieved63,212 (Fig. 3c). Non-adhesive hydrogel surfaces with low friction coefficients have been developed based on the incorporation of various lubricating components, including non-adhesive dangling chains61 (such as for poly(2-acrylamido-2-methyl-1-propanesulfonic acid) hydrogels synthesized on Teflon substrates), uncrosslinked polymers62,213 (such as for porous polyacrylamide hydrogels filled with uncrosslinked alginate) and lipids65 (such as for poly(hydroxyethylmethacrylate) hydrogels with incorporated phosphatidylcholine lipids). In these hydrogels, the lubricating components provide non-adhesive surfaces against the counter surfaces, resulting in highly lubricated interfaces with low friction coefficients.
Acoustic properties
Tissue-matching acoustic impedance is critical to minimize interfacial reflection or scattering of acoustic waves in the acoustic mode214. The acoustic impedance of a homogenous material is determined by the effective density and bulk modulus of the material. Hence, to match the acoustic impedance of biological tissues, the effective density and bulk modulus of the hydrogel interfaces must correspond to those of the target tissue94. Notably, because the effective density and bulk modulus of hydrogels with high water content are almost the same as those of water215, the acoustic impedance is approximately the same as that of water-rich biological tissues93,169,170. For example, hydrogels with a high water content based on polyacrylamide, polyvinyl alcohol, polyacrylic acid and poly(hydroxyethylmethacrylate) have been adopted as acoustic couplants in both academic and clinical settings93,95,216 (Table 2).
Electrical properties
Electrical conductivity is commonly introduced to hydrogels by incorporating electrically conductive phases5. However, if these phases are non-continuous, they cannot effectively transport electrical currents. Hence, the percolation of electrically conductive phases within hydrogel interfaces is needed for high electrical conductivity (Fig. 3d). Various conductive fillers, including metal nanowires217,218 (such as Ag and Au), carbon nanotubes219, graphene19,220 and conducting polymers137 (such as poly(3,4-ethylenedioxythiophene):poly(styrene sulfonate), polypyrrole and polyaniline), have been used to form percolated electrically conductive phases in hydrogel interfaces (Fig. 3d). Notably, hydrogel interfaces purely consisting of percolated conducting polymers have been developed and show very high electrical conductivity77,138,221,222,223.
The capacitance of metallic electrodes is typically low because it originates from the surface-bound electrical double layer5. Low capacitance at the electrode–tissue interface can result in high electrical impedance and inefficient charge injection, which are highly undesirable for electrophysiological recording and stimulation, respectively5,172. Incorporating volumetric capacitance into the hydrogels to overcome the limitation of the surface-bound electrical double layer can help in achieving high capacitance5 (Fig. 3e). To incorporate volumetric capacitance, conducting polymer-based hydrogel interfaces have been developed that can form electrical double layers in the nanoporous structure of the conducting polymers224,225. For example, conducting polymer hydrogels based on poly(3,4-ethylenedioxythiophene):poly(styrene sulfonate) have high volumetric capacitance, low impedance and high charge injection capacity in wet physiological environments, which are required for in vivo electrophysiological modulation77,138,139,223.
Optical properties
The transmittance of light in materials is determined by the degree of scattering and blockage of the incident light within the material. When the size of the hydrogel constituents (for example, the polymer aggregates or crystalline domains) is greater than one-tenth of the wavelength of the incident light, light scattering becomes substantially higher, resulting in decreased transmittance226. Hence, to achieve high transmittance, light scattering must be minimized by limiting the size of the hydrogel constituents. This design principle has been implemented by minimizing the size of phase-separated polymer-rich and water-rich domains141 (such as for polyethylene glycol hydrogels with high molecular weight) and by fabricating nanoscale semi-crystalline domains227 (such as for nanocellulose-reinforced polyvinyl alcohol hydrogels) to achieve hydrogel interfaces with high optical transparency.
Light delivery through optical waveguides relies on total internal reflection, which requires a higher refractive index for the internal medium than for the external medium141. Hence, it is critical to ensure that the refractive index of the hydrogel is higher than that of the target tissue141 or the cladding material141,142,174. The refractive indices of hydrogels are inversely proportional to the hydrogel water content, ranging between the refractive index of pure water (n = 1.331) and that of pure polymers (n = 1.4–1.7)141. Hence, the refractive index can be tuned by controlling the water content of the hydrogel interfaces. For example, step-index hydrogel optical fibres have been developed based on a hydrogel core (such as with polyethylene glycol hydrogels) and cladding (such as with alginate hydrogels) with a varying equilibrium water content142,174.
Chemical properties
The diffusivity of chemicals in hydrogel interfaces (Dhydrogel) is determined by the interactions between the chemicals and the polymer networks of the hydrogel228,229. In particular, the relative size of the chemicals (their radius rs) and of the hydrogel network (the mesh size ξ) significantly affect chemical diffusivity87,145:
where Dwater is the diffusivity of the chemical in water230,231,232.
When the size of the chemical is smaller than the hydrogel mesh size, the chemical can diffuse freely within the hydrogel. Otherwise, the diffusion of the chemical is substantially slowed by steric hindrances from the polymer network. Hence, choosing the mesh size based on the size of the chemical employed for delivery or sensing tunes the diffusivity of the chemical in hydrogel interfaces (Fig. 3f). The mesh size of hydrogels depends on various parameters such as water content and crosslinking density31,32,33,87. To further increase the diffusivity of chemicals, hydrogel interfaces with nanostructures233 (such as poly(amidoamine) dendrimers), microscopic or macroscopic porosity234,235 (such as porous alginate hydrogels), and stimuli responsiveness236 (such as poly(N-isopropylacrylamide)) have also been developed. Several reviews discuss diffusivity in hydrogel interfaces86,87,237,238.
The degradation of hydrogels mostly relies on breaking the network building blocks (for example, polymer chains or crosslinks) and thus losing mass to the surrounding physiological environment. Hence, the biodegradability of hydrogel interfaces depends on the incorporation of biodegradable building blocks181 (Fig. 3g, left). The rate of biodegradation can be controlled by engineering the ratio of degradable building blocks to their non-degradable counterparts. The biodegradation of hydrogel interfaces depends on two major mechanisms: hydrolysis and enzymolysis (Fig. 3g, right). In wet physiological environments, water drives the hydrolysis of ester groups. For example, introducing ester groups in the networks produces hydrolytically biodegradable hydrogel interfaces, such as oxidized alginate239,240 and polyethylene glycol241,242. Hydrogel interfaces containing biopolymers can undergo biodegradation via enzymolysis. Cells and physiological fluids in the human body are rich in enzymes that can cleave and digest various chemical bonds (for example, amide groups) in biopolymers such as collagen31, gelatin189,190, hyaluronic acid243 and chitosan194,195,196.
To achieve a high binding affinity to target chemicals, binding components such as ligands have to be incorporated into the hydrogel interfaces146,175,177,244. Hydrogel interfaces developed for the sensing of diverse chemicals (for example, glucose or reactive oxygen species) or chemical groups in cells (for example, B cells) incorporate the corresponding ligands or active functional groups178,245,246,247,248 (such as glucose oxidase for glucose and T cells for B cells) into the network. Notably, the high binding affinity between drugs and ligands in hydrogel interfaces can also be used for the controlled release of drugs in the human body175 (for example, polyacrylamide hydrogels coupled with the GyrB protein used for the triggered release of vascular endothelial growth factor)121. Several other reviews address the design of hydrogel interfaces for chemical sensing and release176,177,246.
Biological properties
Cells adhere to substrates through the binding of proteins such as of integrins to ligands on the surface249. Hence, incorporating cell-adhesive ligands into hydrogel interfaces provides cell adhesiveness. Because various biopolymers in the extracellular matrix (such as collagen, gelatin or hyaluronic acid) possess integrin-binding ligands, cells can readily adhere to hydrogel interfaces containing these biopolymers31. Specific cell-adhesive proteins (such as fibronectin)250,251 and peptide motifs (such as arginyl-glycyl-aspartic acid (RGD))252,253 have also been incorporated for cell adhesiveness.
To achieve alow foreign-body response, hydrophilic, biomolecule-functionalized, zwitterionic and drug-releasing surfaces have been introduced to hydrogels28,67,188 (Fig. 3h). Hydrophilic surfaces form a hydration layer as a result of bonding with water molecules in wet physiological environments254. The hydration layer acts as a physical barrier against the adhesion of foulants (such as proteins and cells)255. Physical barriers against foulants, based on various surface-functionalized biomolecules such as antimicrobial peptides256,257, have also been introduced to hydrogels. Furthermore, zwitterionic polymers have also been widely utilized to form anti-foreign-body response surfaces75. Poly(phosphatidylcholine), poly(sulfobetaine) and poly(carboxybetaine) are the most commonly adopted zwitterionic polymers that can form hydrogels or that can be grafted to other hydrogel networks66,70,73. Apart from various physical barriers, drug-releasing surfaces have been explored to gradually elute anti-foreign-body response drugs from the surfaces of hydrogels258.
Notably, various biologically important functional groups can be introduced to hydrogel interfaces to facilitate desirable cell or tissue responses (for example, cell proliferation or differentiation) beyond cell adhesion and anti-foreign-body response. Several reviews offer more detailed discussions31,81,183,259.
Future perspectives
Recent advances in diverse applications have shown the great promise of hydrogel interfaces for the bridging of the human body and machines (Fig. 1); however, there are remaining challenges and opportunities for future developments to effectively achieve this. The importance of continued innovations is particularly highlighted by the stark limitations in establishing long-term functional interfaces between implantable devices and the human body. Despite tremendous progress in implantable devices and hydrogel interfaces for therapeutic, diagnostic and assistive applications in recent decades, the loss of function and failure of long-term implants in the human body owing to suboptimal integration with the target tissues, tissue damage, foreign-body response and/or fibrotic isolation of the implants remain unsolved problems4,188. Hence, the development of next-generation hydrogel interfaces should focus on addressing these challenges using multidisciplinary investigations and rationally guided design rules. In this section, we discuss future perspectives based on two conceptual next-generation hydrogel interfaces (Fig. 4a,b).
a | A multimodal hydrogel interface providing multifunctional interfacing between a machine (implanted device) and a target tissue. b | An all-hydrogel machine providing a multifunctional interface to a target tissue. FBR, foreign-body response.
Multimodal hydrogel interfaces
In the human body, internal organs are integrated with one another by connective tissue to form robust, seamless and multifunctional interfaces. The internal organs also maintain anatomical boundaries without adhesion by serous membranes (or serosa) at their outermost surfaces. These characteristics provide inspiration for multimodal hydrogel interfaces for seamlessly integrated, long-term and multifunctional devices.
For epidermal and wearable applications, multimodal hydrogel interfaces can offer multifunctional interfacing between the skin and various sensors and devices. For instance, advanced wound dressings may require hydrogel interfaces with multiple modes of interaction, including mechanical integration to the skin, electrical, optical, acoustic and chemical sensing for wound monitoring, and chemical delivery of therapeutic substances53. For ingestible and minimally invasive applications, multimodal hydrogel interfaces can potentially offer new treatment strategies by synergistically combining various functionalities. For example, low-friction, conductive, transparent and drug-releasing hydrogel coatings on catheters, guidewires or stents may provide electrical and/or optical monitoring of the target tissues and delivery of drugs on top of the conventional clinical treatments offered by minimally invasive medical devices260,261. For implantable applications, anti-foreign-body response hydrogel interfaces around the implanted device may provide a long-term functional interface to the human body, similar to serosa in organs (Fig. 4a). Similar to connective tissue in organs, bio-integrative hydrogel interfaces between the implanted device and the target tissue may provide seamless and multifunctional interfacial integration with the human body.
To achieve multimodal hydrogel interfaces, new hydrogels with improved properties will be required. To minimize the foreign-body response, hydrogels with improved efficacy and longevity in diverse tissue environments must be developed. Bio-integration also requires tissue adhesive hydrogels capable of long-term integration to the target tissue without inflammatory responses and subsequent fibrotic encapsulation. Furthermore, there is a need to address potential tradeoffs between different properties and functional modes of hydrogel interfaces. For example, increasing the electrical conductivity of a hydrogel interface often leads to less favourable mechanical (such as stretchability, fracture toughness and interfacial toughness) and optical (such as transparency) properties5.
All-hydrogel machines
Existing devices are mostly composed of conventional materials, such as metals, plastics and elastomers, that are dissimilar to biological tissues. By contrast, biological tissues and organs (except teeth, nails and bones) are essentially multifunctional structures fully consisting of hydrogels seamlessly integrating cells and extracellular matrices. Hence, biological tissues and organs not only serve as applicational targets but also provide inspiration for all-hydrogel machines (Fig. 4b).
Because of their tissue-like composition and properties, all-hydrogel machines offer promise for the improvement of device biocompatibility. Furthermore, the absence of conventional materials can potentially enable functional devices that are truly transient and tissue-integrative by allowing the ultimate biodegradation and tissue in-growth of the all-hydrogel machine without leaving residual materials. Although all-hydrogel machines may benefit a broad range of applications, they would be particularly advantageous for implantable applications where biocompatibility, degradation and tissue integration are critical requirements207.
The realization of all-hydrogel machines will require the development of hydrogels with new and improved properties. For example, conductive hydrogels with improved electrical properties (such as electrical conductivity and capacitance) and hydrogel waveguides with optimized optical properties (such as transmittance and refractive index) must be developed to successfully replace existing metal-based electrodes and stiff optical waveguides, respectively. Similar to multimodal hydrogel interfaces, bio-integration and an improved anti-foreign-body response will be critical to ensuring the long-term functional preservation of all-hydrogel machines in physiological environments. Hydrogels with new properties, such as electrically semiconducting and insulating properties, will require novel materials to replace existing silicon-based semiconductors and plastic-based or elastomer-based insulators, respectively. Furthermore, synergistically with material developments, new and advanced fabrication strategies should be developed to manufacture future all-hydrogel machines. While various advanced fabrication methods, such as photolithography and additive manufacturing, have been utilized for individual hydrogel materials, manufacturing of all-hydrogel machines consisting of a broad range of hydrogels with diverse functionalities in a highly integrated and precise manner would necessitate further improvement of existing methods and development of new fabrication strategies.
Overall, hydrogel interfaces will play an essential role towards the futuristic vision of a seamless merger of machines and humans. Despite a promising outlook, hydrogel interfaces will undoubtedly face numerous scientific, engineering and translational challenges during future development and implementation. However, in our wildest imagination, hydrogel interfaces may one day allow us to blur the boundary between biological and abiotic systems and may enable future human–machine hybrids.
Recommend
About Joyk
Aggregate valuable and interesting links.
Joyk means Joy of geeK